Microfluidics ? Key Concepts and Applications - Part 1
June 07, 2019
Story
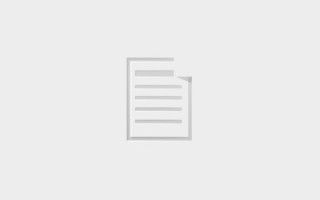
Life science and biotech companies work with semiconductor material suppliers and microfabrication foundries throughout the course of the development, production and commercialization of products.
Microfluidics is the science and engineering of controlling fluids in extremely small volumes, usually in the range of microliters to picolitres. The fluids are typically held in networks of channels with dimensions ranging from 1 to 500 μm, contained within what is known as a microfluidic device. Microfluidic components or instruments, e.g. pumps, transfer fluids through inlet and outlet ports into the device.
At such small dimensions, fluids exhibit quite different behaviors from those that we are familiar with, and capillary action can dominate their movement. Fluids are allowed to move through a microchannel network, which typically includes channels, chambers, reservoirs and additional features, such as measuring, sensing and actuation components. The size and geometry of the microchannels vary depending on the desired function and application, which might include mixing, separation, filtration, detection, heating and droplet creation.
Microfluidics is a broad field with many different applications in the following areas:
- Molecular and cell biology research
- Genetics
- Fluid dynamics
- Micro-mixing or separation
- Diagnostics
- Tissue engineering
- Drug delivery
- Fertility testing and assistance
- Environmental analysis
- Synthesis of chemicals or proteins
Many of these applications require specific fabrication techniques and material properties. In most cases, microfluidic devices also require post-fabrication modifications such as applying treatments or coatings to the surface. This can increase their repulsion or attraction of water (known as hydrophobicity or hydrophilicity). These modifications can also include the integration of reagents or specific molecules or cells in the devices, as well as the inclusion of electrodes, magnets or optical components. Microfluidic devices often require post-manufacturing assembly into holders or cartridges, predominantly made of plastic.
Microfluidics in Biotechnology
Microfluidics is a multidisciplinary field in which collaboration between chemists, physicists, biotechnologists, doctors and different engineering disciplines is needed. This can include everything from the conceptualization and development of ideas, to the design, manufacturing and testing of the microfluidic devices.
Biotechnology is the sector that commercially utilizes the greatest number of microfluidic devices. In this field, life science and biotech companies need to work with semiconductor material suppliers and microfabrication foundries throughout the course of the development, production and commercialization of their products.
Microfluidic devices are being increasingly used for biological applications, because precise and controlled experiments can be conducted at a lower cost and faster pace, directly at the point of use and with a higher throughput than is possible with conventional laboratory techniques. Moreover, in microfluidics, we can much better mimic what is happening in living organisms, and more precisely control the fluid flow, mixing, pressure and environment than with traditional analytical methods.
There are multiple advantages to microfluidic devices. Their small size enables lower costs of production and materials used in the devices. Moreover, the small number of reagents used to achieve the same results drastically reduces operating costs, while the same number of cells and samples enable more test repetitions, and hence enable much more precise diagnostics. The power consumption required to run analysis procedures is also reduced. Furthermore, microfluidic devices are more practical for use in remote locations such as developing countries, where the spread of infectious diseases is a problem, and where there is limited access to clinical labs or equipment for testing.
In summary, some of the advantages of using microfluidic devices are:
- Reduced volumes of samples, reagents and waste
- Decreased cost of testing and procedures
- Faster results
- Improved automation and portability
- More precise quantitative results and real-time monitoring
Materials
At the micro scale, the total surface area-to-volume ratio is greatly increased, therefore the properties of the material in which these devices are built are far more important than at the macro-scale. Consequently, significantly more attention must be paid to the chosen materials and their surface properties.
The materials most commonly used to build microfluidic devices are glass, silicon and polymers, each with their specific benefits and drawbacks. The material should primarily be chosen on the basis of its properties, compatibility with blood or treatments, and the requirements of the intended application. Subsequently, the material needs to be evaluated based on fabrication options and their respective costs.
The choice of material should be evaluated for each individual product development stage, knowing that the options selected during prototyping might not be scalable to high volume manufacturing later, and therefore, different manufacturing options may subsequently be necessary. For example, during the development and prototyping stages, the performance of the device risks being compromised by ease of manufacturing, whilst in the production stages, performance reliability and costs are the most important factors.
Silicon is the most common material used for microfluidic applications because of its well-known properties and fabrication techniques. Some of the properties of silicon that are attractive for microfluidic devices are excellent resistance to organic solvents, high thermo-conductivity and thermo-stability, rigidity, and ease of metal deposition. Silicon is extremely well understood, since its production and operation processes are adapted from the MEMS (Micro Electro Mechanical Systems) and semiconductor industry.
However, using silicon is expensive, and requires clean room facilities with specialized equipment and trained personnel. Also, silicon is opaque, which limits its use in applications where transparency of the device for imaging or fluorescence detection is required. Its rigidity can also be a drawback when active components such as valves and pumps need to be integrated into the device.
Glass has many of the same advantages as silicon, while also being transparent, biocompatible, and exhibits a low non-specific surface absorption of molecules. As with silicon, in addition to the requirement of cleanroom environments and high fabrication costs, the difficulty of integrating additional components limits the utility of glass for certain microfluidic applications (Fiorini and Chiu, 2005).
Glass and silicon devices are easily metalized and their surfaces can be modified to prevent non-specific absorption of molecules and for increased wettability. They are ideal for capillary electrophoresis (CE), organic synthesis, droplet formations, DNA sequencing and PCR microfluidic applications, amongst others (Ren et al., 2013).
Over the last few decades, the use of polymers for microfluidic devices has developed and polymers have become a more cost-efficient material compared to silicon and glass. Polymers offer the advantages of transparency, low cost of material and far simpler integration of active components such as valves or pumps.
There are many polymers, each with different properties. They can be divided into three primary categories: elastomers, thermoplastics and thermosets.
Elastomers, such as polydimethylsiloxane (PDMS), are widely used for research and rapid prototyping because of their ease of fabrication, ease of integration of multiple layers and active components, and satisfactory optical transparency. Furthermore, PDMS is well suited for long-term cell culture applications because of its gas permeability. PDMS, however, is incompatible with organic solvents and permits absorption of small hydrophobic and biomolecules in the channel walls. In addition, the low elastic modulus of PDMS, which helps with the integration of components, also makes it prone to deformation and breakage of channels and features.
In terms of fabrication, multiple PDMS devices can be cast from a single silicon or glass mold, manufactured using MEMS techniques. As with silicon and glass, surface modifications can be made to obtain specific surface properties such as non-absorption of molecules and wettability, although these modifications are not as durable in PDMS (Nge et al., 2013).
Thermoplastic polymers such as polystyrene (PS), polycarbonate (PC), and polymethylmethacrylate (PMMA) are materials that when heated to their glass transition temperature (Tg) can be formed into specific shapes by cooling; and if desired, they can then be re-heated and re-shaped.
Different thermoplastic polymers have different properties, but in general, they are all transparent, cheap (thus mostly used as disposable devices), resistant to permeation of small molecules, and more rigid than elastomers.
Some of the fabrication techniques for thermoplastic chips include microinjection molding, hot embossing, imprint-lithography, solvent imprinting, and sometimes micromachining, amongst others. These fabrications techniques, except for machining, require the manufacture of a mold, which is very expensive, and therefore these processes are more suited for large volume production rather than prototyping.
Thermoplastics are not applicable when high temperatures, organic solvents or high aspect ratios are needed in the devices and applications. The bonding of thermoplastic polymers is troublesome because fusion-thermal bonding, which is bonding at the glass transition temperature (Tg), is not as strong as the other bonding techniques used for silicon, glass and PDMS devices, and can also alter the features of the microchannels.
Thermosets are also transparent, but irreversibly shaped. Some examples include thermoset polyester (TPE), and SU-8 photoresist and polyimide, which often serve as negative photoresists. When compared to thermoplastics, thermosets have better stability when subjected to organic solvents and higher temperatures, but they are not as widely used for microfluidic applications because of their higher cost.
Table 1 summarizes the most important characteristics of these materials for the fabrication of microfluidic devices. To maximize performance and potential applications, hybrid microfluidic devices can be designed by combining various materials. This should, of course, be done in such a way that the second material does not compromise the advantages provided by the first material.
Characteris-tic |
Silicon |
Glass |
Thermoplas-tics |
Thermosets |
Elasto-mers |
Transparen-cy |
No |
High |
Medium to high |
High |
High |
Strength (modulus) |
High |
High |
Low |
Low to medium |
Very low |
Thermal stability |
Very high |
Very high |
Medium |
High |
Medium |
Solvent compatibili-ty |
Very high |
Very high |
Medium to high |
High |
Low |
Material cost |
High |
High |
Low |
Medium |
Medium |
Fabrication |
Photolithography, etching, etc. |
Thermo-molding |
Casting or photo-polymerization |
Casting |
|
High-aspect ratios obtainable at the micro and nano scales, and multifunctional layering. |
Not suitable for prototyping. Low manufacturing costs at mass production scale. |
Not suitable for mass production. |
Not suitable for mass production. Poor resolution. |
||
Smallest channel dimension |
<100nm |
~100nm |
<100nm |
<1μm |
Table 1. Summary of properties of materials for microfluidic device fabrication (Ren et al., 2013)
As previously mentioned, materials should be chosen based on the surface and material properties needed for each application, and then based on cost and manufacturing options. As an example, even though polymer microfluidic devices will have a lower manufacturing cost at production scale, when compared to silicon or glass, not all the applications can be supported by polymer devices. Silicon or glass needs to be chosen in applications requiring high temperatures and organic solvents. Moreover, not all the structural requirements – e.g. high-aspect ratio features, patterning of metals, high quality and good dimensional control – are amenable for polymer devices.
In summary, the decision on which materials to use is driven not solely by costs, but mainly by the specific properties needed for each application. When evaluating a material, however, the expected production volume and the production scale options for fabrication should also be considered, since they will influence design, cost and quality.
Marisel De Jesús Vega, Program Manager at Sensera Inc., received her B.S. from University of Puerto Rico, Mayagüez campus and her Doctoral degree from University of Massachusetts Lowell, both in Chemical Engineering. Her interests are microfluidic devices, microfabrication and materials.