Why the Properties of Graphene at Cryogenic Temperatures are Making Quantum Computing Increasingly Viable
May 12, 2021
Story
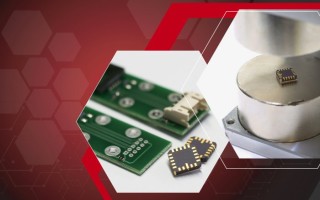
Quantum computing is the ultimate hardware accelerator.
According to pioneers such as IBM, in the not-too-distant future we can expect quantum computers to do a lot of the heavy lifting that traditional transistor-based computers struggle with today. A good example would be modelling complex systems or providing the processor power needed to train large neural networks. IBM’s quantum computing roadmap has a target of achieving a 1,000 quantum bit (qubit) machine by 2023.
There are examples of processing tasks already handled by specialized hardware. Graphics processing, for example, is accelerated through dedicated silicon for both image processing and display rendering. However, researchers expect quantum computers to be used in very different ways compared to a conventional hardware accelerator. By way of a simple comparison, we can think of quantum computers as being as superior to today’s multicore processors with hardware accelerators, as a modern PC is superior to a valve-based computer.
What conventional electronic circuits, of all types and at all levels of complexity, have in common, is their physical nature. It is relatively easy to observe electrons and to store a value represented by electrons in a physical material or device. In the quantum world, things are not quite so simple.
The quantum world is an interesting place, instead of just on and off states, exotic terms like superposition, entanglement, and decoherence are used to describe what goes on at a quantum level.
To put it in an overly simple way, superposition means a qubit, can represent several states at the same time. This could be likened to a multilevel flash cell, that uses 4 bits to represent any number between 0 and 15, representing all 16 states at the same time. Things get even more interesting when we talk about entanglement, which refers to two qubits that are ‘quantum’ connected. This means whichever one of the 16 states our first qubit represents at any given time, will be replicated by the second qubit simultaneously, even though it is physically separate from the first qubit.
Quantum measurement becomes even more challenging in the real world because quantum systems need to be isolated from any unwanted external influence, that can cause the quantum effects to stop working. known as decoherence. This is where quantum computing starts to get complex. For any computer to be useful we must be able to observe the results of computations, and decoherence makes this task a lot harder.
The Role of Graphene Hall Effect Sensors in Observing Qubits
Manipulating qubits to enable quantum computations is difficult and usually achieved using microwaves and/or vector magnets. Achieving close control of qubit states, to avoid occurrences such as decoherence, is key to delivering robust, effective quantum computing. However, somewhat advantageously, observing the superposition state of qubits when they are entangled without inducing decoherence, is less difficult if the entire structure is operated at temperatures approaching absolute zero.
Utilizing vector magnets to control the qubit state means that magnetic fields and quantum computing are inextricably linked. Vector magnet devices use electrically controlled magnetic fields to manipulate qubits and achieve the desired state. This approach to quantum computing is still in its infancy and being studied widely. Working at the quantum level means that even very small interferences can have significant effects on the system. For example, the earth’s magnetic field is a considerable challenge to the efficient operation of vector magnet-controlled quantum computers. To tackle this problem, the control volume — where the qubits are located — is magnetically shielded to cancel out the effects of external fields.
Therefore, to achieve the highest performance quantum computer, with maximum efficacy and minimum decoherence, requires magnetic shielding that can exist and operate at very low temperatures. Such essential close performance monitoring, measurement, and control is extremely challenging at cryogenic temperatures, and very difficult for conventional sensing devices.
This is where the Paragraf graphene-based Hall effect sensor (GHS) comes into its own. By employing graphene as the active material, the GHS operates with very high accuracy at temperatures all the way down to absolute zero.
Less Power Dissipation When Operating at Cryogenic Temperatures
Paragraf’s GHS technology has proven its ability to operate at cryogenic temperatures and provide accurate, repeatable measurements with high resolution and sensitivity. This is crucial since other Hall effect sensors targeting this application area are much less sensitive, therefore needing considerably more power to amplify their smaller signal output.
The increased power requirement causes significant heat to be generated, which in most applications is not a problem. But when the application requires temperatures approaching absolute zero, any excess thermal energy becomes problematic. Standard Hall effect sensors require many mA’s of power to operate, generating considerable heat. By comparison, Paragraf’s GHS only needs nA’s of current and dissipates pW levels of power, six orders of magnitude better than other cryogenic magnetic sensors.
The GHS also boasts remarkable range capabilities. At room temperature, the sensors can measure fields of over 9 T. Even more significantly, sensitivity increases as the temperature decreases, more than doubling in the drop from room temperature to 1.8 K.
GHS sensors have been extensively tested across magnetic fields of -1 T to 9 T, at various temperatures below 50 K. Results have demonstrated very high sensitivities across the range, showing that the technology’s applicability can map and calibrate magnets in quantum computing applications. Importantly, the sensor’s behaviour is precise, repeatable, and stable, through repeated temperature cycling from high (300 K) to low (1.8K).
Paragraf’s sensor returns measurement resolutions in the sub-ppm range, far beyond the performance of conventional Hall effect sensors.
Graphene continues to prove itself to be an amazing material. In many respects, the way we exploit it will be just as varied and significant as the way we use quantum computing in the future. Thanks to its ability to function at cryogenic temperatures, with incredible performance, that day may be sooner than expected.
For more information about the Paragraf graphene Hall-Effect sensor series, please visit: https://www.paragraf.com/graphene-hall-effect-sensors/